|
/ Home
/ Facilities
Facilities
The energy scale depicts the operating range for each spectrometer. All
instruments are capable of sample characterization from liquid helium
temperatures to room temperature. Please scroll down to read more about
each instrument.
Fourier Transform Spectroscopy
A Fourier transform spectrometer is a Michelson interferometer,
invented by Albert A. Michelson in 1880, which utilizes a movable
mirror and a broad-band source. With this instrument, Michelson
conceived the basic techniques of Fourier transform spectroscopy. He
measured intensity levels by eye and made crude estimates of the
spectrum of the Zeeman effect. To compute Fourier transforms, he
invented a mechanical sine and cosine analog computer, which could
handle about 80 data points. Using the techniques available to him,
Michelson resolved several spectra, which were doublets.
The main component in the Fourier Transform
Infrared spectrometer is an interferometer. This device splits and
recombines a beam of light such that the recombined beam produces a
wavelength-dependent interference pattern or an interferogram. The
Michelson interferometer is most commonly used.
A Michelson interferometer consists of two mirrors
and a beamsplitter positioned at an angle of 45 degrees to the mirrors.
A KBr beamsplitter coated with Germanium is typically used for mid IR
region and Mylar for the far-infrared (terahertz) region. Incident
light strikes the beamsplitter so that half of the light is transmitted
through the beamsplitter and half is reflected to the mirrors. The two
components are then reflected back and recombined at the beamsplitter
with half of the light passing on toward the sampling areas and half
traveling back toward the source. The signal at the detector is a
cosine wave. In general, the function of the interferometer is to
disperse the radiation provided by the IR source into its component
frequencies. With polychromatic light (radiation with more than a
single wavelength), the output signal is the sum of all the cosine
waves which is the Fourier Transform of the spectrum or an
interferogram. The interferogram contains the basic information on
frequencies and intensities characteristic of a spectrum but in a form
that is not directly interpretable. Thus, the information is converted
to a more familiar form, a spectrum, using Fourier Transform methods.
|
Grating
Spectrometer
A grating monochrometer is utilized for near normal reflectance
measurements over an energy range from 14000 to 50000cm-1. The
spectrometer is fitted with a cryostat and temperature measurements may
be performed from liquid helium temperatures to over 600 Kelvin.
In-situ gold or aluminum coating of the samples permits absolute value
measurements. |
Martin-Puplett
Specially designed to operate in the near-millimeter and far-infrared
region of the frequency spectrum. One of the most important features of
these instruments is the inclusion of polarizing grid beam-splitters.
The efficiency of the grid beam-splitters is close to 100% for all
wavelengths up to the cutoff determined by the grid separation (4µm).
The polarizer is used both as a polarizer and as analyzing chopper.
The instrument operates under vacuum to avoid
water vapor absorption and microphonism. The optics platform is
"floating" inside the vacuum box to avoid changes in alignment. The
standard light source is a high pressure mercury lamp with a DC
stabilized power supply.
A unique capability of our instrument is the
ability to perform absolute measurements over temperatures from liquid
helium to room temperature. Additionally characterization of micron
sized samples is possible.
|
NIR-Visible Variable Angle
Spectroscpic Ellipsometry (VASE)
Ellipsometry is a technique whereby the amplitude and phase of the
reflected light can be measured simultaneous. This powerful tool was
originally invented by Paul Drude in 1887, which utilizes a two
polarizers to measure the complex ratio of light reflected normal to
and in the plane of incidence. Drude was interested in examing the
optical properties of materials to test his classical theory of
electron dynamics in solids. He not only derived the equations of
ellipsometry, but carried out the first measurements on a number of
transparent and opaque materials.
Since the days of Drude ellipsometry has become a
powerful tool for accurately measuring the optical constants in a
timely manner.The technique currently used was originally developed by
Aspnes in 1975. He initially followed a similar procude as Drude.
Specifically light from a monochrometer is sent through a polarizer
fixed at fourty five degrees from the plane of incidence. This light is
then reflected at a large angle of incidence from the sample. The
reflected light then passes through another polarizer (the analyzer),
and finally is focused onto the detector. Aspnes realized that by
rotating the analyzer while the polarizer remained fixed, the signal
would be modulated and therefore the optical constants could be
determined from the phase and amplitude of the detector signal. This
technique has since seen some mild improvements including the
incorporation of a "compensator". This element delays the phase of one
polarization with respect to another, resulting in circularly polarized
light. By combining a rotatable compensator and a rotatable polarizer
and elliptical polarization can be attained, allowing one to measure
the optical constant with extreme accuracy.
Besides allowing one to measure the phase as well
as the amplitude of the reflected wave, Ellipsometry is also an
extremely surface sensitive technique. Therefore it is often used to
determine structural information of multilayered samples, as well as
the quality of interface between two materials. However this also means
that measurements at low temperatures must be performed under
Ultra-High Vacuum(UHV). We have designed and implemented a UHV chamber
as an addition to our commercial VASE that allows us to make
measurements from 30-400K at pressures as low as 1.4*10^-10 mBarr.
|
s-SNOM
Scattering type Scanning Near-field Optical Microscopy allows for
nanoscale imaging at IR wavelengths. s-SNOM is useful for studying
variations in optical properties on lengthscales too small to discern
with conventional, diffraction limited, IR spectroscopy. One example is
the imaging percolating metalic clusters during the metal to insulator
transition of Vanadium Dioxide [1, Figure below].
Principle:
The lighting rod effect of a sharp metallic tip concentrates the
incident IR radiation to a volume of roughly ~a^3, where a is the tip's
radius of curvature [Figure below]. The radius of curvature for a
commercial metallized AFM tip can be as small as ~10nm. Due to the
lightning rod effect being wavelength independent the sharpness of the
tip sets the fundamental resolution limit rather than the wavelength.
Various incarnations of the s-SNOM have demonstrated ~10-100nm
resolution for wavelengths ranging from visible all the way to
microwave [2,3,4]. s-SNOM images exhibit wavelength dependent contrast
which allows for material identification much in the same way that an
FTIR spectrum does. The origin of the contrast at such small
lengthscales is most simply explained by a model where the tip and
sample interact through dipole-dipole near fields. A sample having a
strong resonance (for instance SiC at ~930cm-1) can have a large dipole
induced under the tip. Resonant enhancement of the scattered light has
been demonstrated for SiC to exceed the scattering from even a perfect
conductor such as Gold [2]. Non-resonant contrast is also observed
between metallic and insulating regions such as the metal to insulator
transition in VO2 [1, Figure below].
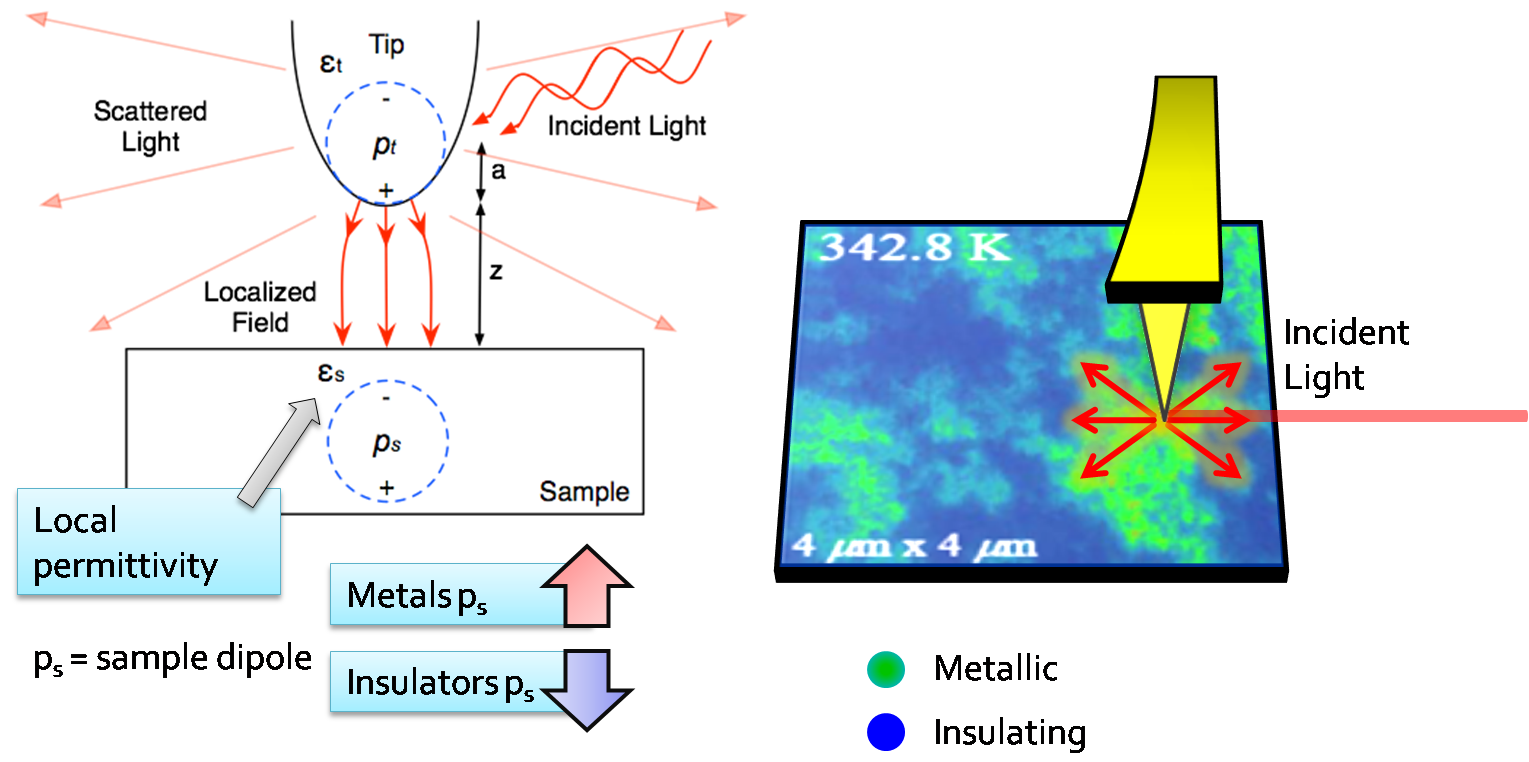
Neaspec sSNOM:
Neaspec sSNOM is a commercial sSNOM presented by
Neaspec GmbH in Germany. The image below shows our Neaspec system setup
that allows infrared nano-imaging and nanoscopy with either
single-frequency lasers (with heterodyne inteferometer) or broadband
source (with Fourier inteferometer).
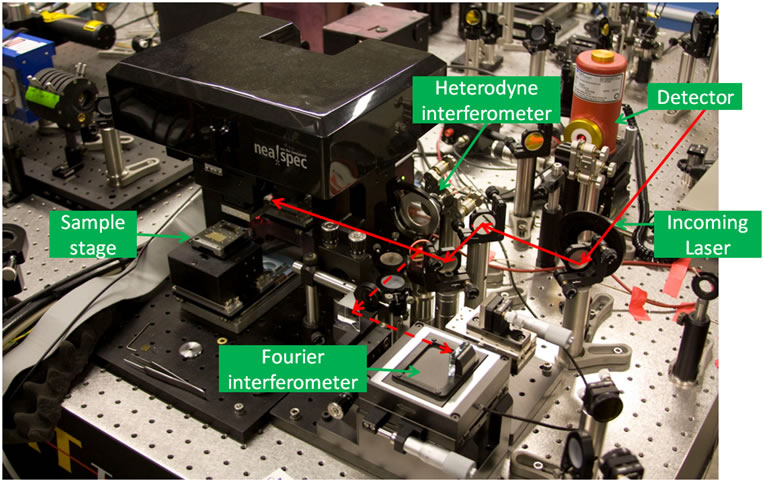
Infrared Sources:
Our infrared sources for the
s-SNOM system include broadband laser source (left image below) and QCL
laser sources (right image below). The broadband infrared source covers
frequency range from 750-2200 cm-1 that is
perfect for infrared nanoscopy. While the QCL lasers offer powerful
(>100 mW) laser output that is perfect for infrared
nano-imaging. The center frequencies of the four QCL lasers shown below
are 1120, 2200, 1600, and 1200 cm-1 (from left
to right), and their bandwidths are around 100 cm-1.
The broadband infrared source and the QCL lasers allow us to explore
wide range of fundamental electronic and optical phenomena such as
metal-insulator transition of transition metals, surface optical phonon
of polar dielectric material, surface plasmon of graphene, etc.
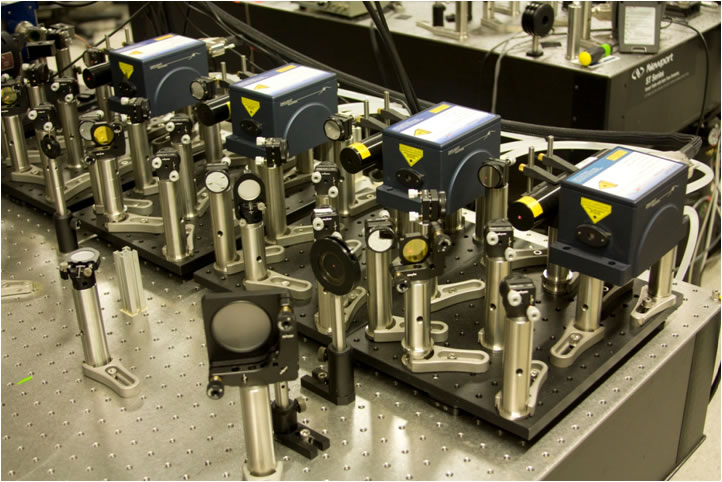
|
References:
1. Z. Fei, G. O. Andreev, W. Bao, L. M.
Zhang, A.S.McLeod, C. Wang, Z. Zhao, G. Dominguez, M.
Thiemens, M. M. Fogler, M. Tauber, A. Castro-Neto, C. N. Lau, F.
Keilmann, D. N. Basov. “Infrared Nanoscopy of Dirac Plasmons at the
Graphene-SiO2 interface” Nano Lett. 11(11), 4701-4705 (2011). PDF
2. Qazilbash, M.M., et al., Mott Transition in VO2
Revealed by Infrared Spectroscopy and Nano-Imaging. Science, 2007.
318(5857): p. 1750-1753. link
3. Hillenbrand, R., T. Taubner, and F. Keilmann, Phonon-enhanced
light-matter interaction at the nanometre scale. Nature, 2002.
418(6894): p. 159-162. link
4. Hillenbrand, R. and F. Keilmann, Material-specific mapping of
metal/semiconductor/dielectric nanosystems at 10 nm resolution by
backscattering near-field optical microscopy. Applied Physics Letters,
2002. 80(1): p. 25-27. link
5. Knoll, B., et al., Contrast of microwave near-field microscopy.
Applied Physics Letters, 1997. 70(20): p. 2667-2669. link
|
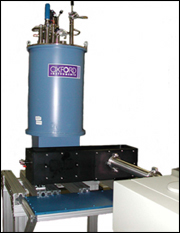
Magneto-Optics
The system is capable of broadband frequency domain spectroscopy in the
range 180GHz to 750THz at cryogenic temperatures. The reflection unit
(black box on right) couples both the Martin-Puplett and the Michelson
interferrometer interferometers (depicted above) to a 9 Tesla
superconducting split coil magnet. Additionaly the unit is designed to
work with various detectors, including thermal bolometers and
semiconducting detectors. The optical layout utilizes an intermediate
focus while preserving optical f/#'s throughout enabling DC magnetic
field measurements of small crystals with polarized light in both
Voight and Faraday geometries. For transparent samples, simultaneous
reflection and transmission can be performed.
|
|